Oxygen is a member of the chalcogen group (Group 16) of the periodic table and
is a highly reactive nonmetallic element and oxidizing agent that readily forms
compounds (notably oxides) with most elements. By mass, oxygen is the third-most
abundant element in the universe, after hydrogen and helium. At standard
temperature and pressure, STP, two atoms of the element bind to form dioxygen,
O2, a diatomic gas that is colourless, odourless, and tasteless.
Discovery
Oxygen was discovered independently by
Carl Wilhelm Scheele,
in Uppsala, Sweden in 1773 or earlier, and
Joseph Priestley
in Wiltshire, UK in 1774, but Priestley is generally given the credit since
his work was published first. The name oxygen was coined in 1777 by
Antoine Lavoisier,
whose experiments with oxygen helped to discredit the then-popular
phlogiston theory
of combustion and corrosion. Its name derives from the Greek roots
οξυζ oxys, "acid", literally "sharp", referring to the
sour taste of acids and -γενης genes
meaning "creator", because at the time of naming, it was mistakenly thought
that all acids required oxygen in their composition.
There are 2 main allotropes of oxygen although a third has recently been
shown to form under high pressures.
Some allotropes of oxygen
|
|
|
O2 - dioxygen
|
O3 - ozone
|
O8 - red oxygen
|
Dioxygen - O2
The common allotrope of elemental oxygen is often just called
oxygen, O2, but to help distinguish it from the element may be
called dioxygen or molecular oxygen. Elemental oxygen is most commonly
encountered in this form, as about 21% (by volume) of the Earth's atmosphere is
O2, the remainder largely being dinitrogen, N2.
At STP, dioxygen is a colourless, odourless gas,
in which the two oxygen atoms are chemically bonded to each other giving
rise to two unpaired electrons occupying two degenerate molecular
orbitals. The electron configuration of O2 molecules in
this form, a diradical, indicates that they should be paramagnetic. This is
a classic example of where a simple Lewis structure fails to account for the
properties and where an MO approach correctly provides the explanation.
The electron configuration (ignoring 1s orbitals) is:
(σ2s)2 (σ2s*)2
(σ2p)2 (π2p)4
(π2p*)2 and from this the Bond Order is
found to be ½(2 -2 + 2 + 4 -2 ) = 2 that is, a double bond as shown in the
Lewis structure as well. The difference though is that the Lewis structure
does not predict the molecule to be paramagnetic. The bond length is 121 pm
and the bond energy is 498 kJmol-1.
A video clip showing liquid dioxygen being poured between the faces
of a magnet and attracted into the magnet field has been prepared as a
Harvard Natural Sciences
Lecture Demonstration.
With 2 electrons to be placed in 2 degenerate orbitals, a number of variations
are possible and the arrangement above where the 2 electrons are
parallel is considered to be the most stable. Note that the spin multiplicity
is given by the formula, 2S+1 and so for S=1 from s=½ + ½
then 2S+1 = 3 i.e. a
spin triplet.
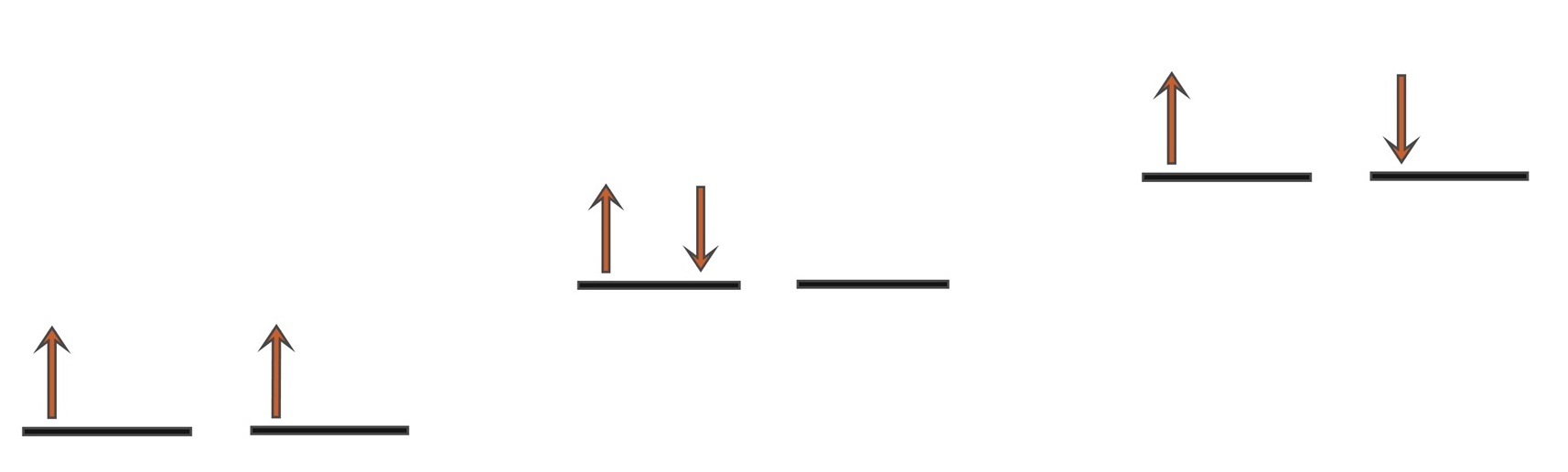
a) e
-s parallel (triplet
3Σ
g),
b) 2 e
-s in 1 orbital (singlet
1Δ
g),
c) e
-s opposed (singlet
1Σ
g)
Singlet oxygen
is the name commonly used for the electronically excited state
shown in b) and it is less stable than the normal triplet state a) by 94.7
kJmol-1. In isolation, singlet oxygen can persist for over an hour
at room temperature. The other singlet state at 157.8 kJmol-1
shown in c) is very short lived and relaxes quickly
to b). Because of differences in their electron shells, singlet and triplet
oxygen differ in their chemical properties. Singlet oxygen is highly reactive.
Reactions of triplet dioxygen are restricted by conservation of spin state
rules and at ambient temperatures this prevents direct reaction with all but
the most reactive substrates, e.g. white phosphorus. At higher temperatures,
or in the presence of suitable catalysts, reactions proceed more readily.
For instance, most flammable substances are characterised by an
autoignition temperature above which they will undergo combustion in air
without an external flame or spark.
The energy difference between the ground state and singlet oxygen is
94.7 kJmol-1 which would correspond to a transition in the near-infrared
at ~1263 nm. In the isolated molecule, this transition is strictly forbidden
by spin, symmetry and parity selection rules, making it one of nature's
most forbidden transitions. In other words, direct excitation of ground
state oxygen by light to form singlet oxygen is very improbable. As a
consequence, singlet oxygen in the gas phase is extremely long
lived (72 minutes). Interaction with solvents however, can reduce the
lifetime to microseconds or even nanoseconds.
Various methods for the production of singlet oxygen exist. A photochemical
method involves the irradiation of normal oxygen gas in the presence of
an organic dye as a sensitizer, such as methylene blue. Singlet oxygen can
be produced chemically as well. One of the chemical methods is by the reaction
of hydrogen peroxide with sodium hypochlorite. This is convenient in small
laboratories and for demonstrative purposes:
H2O2 + NaOCl → O2(1Δg) + NaCl + H2O
In photosynthesis, singlet oxygen can be produced from the light-harvesting
chlorophyll molecules. One of the roles of carotenoids in photosynthetic
systems is to prevent damage caused by any singlet oxygen that is produced by
either removing excess light energy from chlorophyll molecules, or quenching the
singlet oxygen molecules directly.
Biological considerations
Molecular dioxygen is a potentially strong oxidizing agent, based on its
position in the electrochemical series. The standard redox potential is:
O2 + 4H+ + 4e- ⇄ 2 H2O
E⦵ = +1.229 V
which is comparable to potassium dichromate (1.33 V). Nevertheless, reactions
of dioxygen with most substrates tend to proceed very slowly at room
temperature, in the gas phase or in solution. This relative inertness
(unexpected considering it is a diradical) is critical for sustaining
life in a dioxygen atmosphere. Perhaps one of the reasons for this stablilty
is that the reaction above shows that a 4 electron change is required and
this is highly improbable so that it is more likely that a sequence of steps
involving 1 electron changes actually takes place.
O2 + e- → O2-* (superoxide)
O2-* + e- + 2H+ → H2O2 (peroxide)
H2O2 + e- + H+ → OH* (hydroxy radical)
OH* + e- + H+ → H2O (water)
The first step, that generates
superoxide, has an adverse potential of
-0.32 V (ΔG⦵= +30.9 kJmol-1) which imparts
some kinetic stability to the molecule.
Note that both dioxygen and superoxide ion are free radicals that exhibit
paramagnetism. Species like superoxide, peroxide and the hydroxy radical are far too
reactive to be allowed to accumulate in living systems and the primary
defence of the cell appears to be to destroy them as soon as they are formed.
This is done by a variety of enzymes including superoxide dismutase (SOD)
and catalases. Parts of the immune system of higher organisms, however, create peroxide,
superoxide, and singlet oxygen to destroy invading microbes. Reactive
oxygen species also play an important role in the hypersensitive response
of plants against pathogen attack.
Another issue with dioxygen is its low solubility in water at room temperature
and atmospheric pressure. Dioxygen is more soluble in water than is dinitrogen.
Water in equilibrium with air contains approximately 1 molecule of dissolved
O2 for every 2 molecules of N2, despite the
atmospheric ratio of approximately 1:4. The solubility of oxygen in water
is temperature-dependent, and about twice as much (14.6 mgL-1) dissolves
at 0 °C than at 20 °C (7.6 mgL-1). At 25 °C and 1 standard
atmosphere (101.3 kPa) of air, freshwater contains about 6.04 milliliters (mL)
of dioxygen per liter, whereas seawater contains about 4.95 mgL-1.
At 5 °C the solubility increases to 9.0 mgL-1 (50% more than
at 25 °C) for water and 7.2 mgL-1 (45% more) for sea water.
The oxygen in water is unavailable to mammals so that divers (and diving
mammals such as whales and seals) are entirely dependent on the oxygen
carried in the air in their lungs or their gas supply. For divers this is
complicated since at higher partial pressures oxygen can cause acute toxicity
leading to convulsions. Scuba (self-contained underwater breathing apparatus)
divers using compressed air are restricted to
diving above 30 m. If the diver descends to depths near 100 m they can
become unconsciousness from nitrogen narcosis and death usually results.
A dive to 30 m for 20 minutes puts the scuba diver at risk of nitrogen
narcosis and decompression illness. By contrast, the elephant seal can dive
to 1 km for 1 hour without risk of either condition.
Respiratory pigments are capable of fixing dioxygen from the atmosphere,
transporting it to the reacting site and then releasing it. In addition they
are able to counteract small fluctuations in supply or demand by storing dioxygen.
O2 can bind to a single metal center either "end-on"
(η1-)
or "side-on" (η2-). In Haemoglobin and Myoglobin it is "end-on".
|
fully oxygenated Haemoglobin
(with 4 Fe porphins and O2's highlighted)
|
Iron based proteins like
haemoglobin and myglobin as well as copper
based proteins like haemocyanin perform this role in mammalian systems
and lobsters, etc.
Molecular dioxygen, O2, is essential for cellular respiration
in all
aerobic organisms. Oxygen is used in mitochondria to help generate
adenosine triphosphate (ATP) during oxidative phosphorylation. The reaction
for aerobic respiration is essentially the reverse of photosynthesis and
is simplified as:
C6H12O6 + 6 O2 →
6 CO2 + 6 H2O ΔH= -2880 kJmol-1
In vertebrates, O2 diffuses through membranes in the lungs and into red
blood cells. Haemoglobin binds O2, changing its colour from bluish red to
bright red (CO2 is released from another part of haemoglobin through
the Bohr effect). Other animals use
haemocyanin (molluscs and some arthropods) or
haemerythrin (spiders and lobsters). A liter of blood can
dissolve 200 cm3 of O2.
Ozone is colourless or slightly bluish gas (blue when liquified),
slightly soluble in water and much more soluble in inert non-polar solvents
such as carbon tetrachloride or fluorocarbons, where it forms a blue solution.
At 161 K (-112 °C), it condenses to form a dark blue liquid. It is
dangerous to allow this liquid to warm to its boiling point, because both
concentrated gaseous ozone and liquid ozone can detonate.
Most people can detect about 0.01 μmol/mol of ozone in air where it has a
very specific sharp odour somewhat resembling chlorine bleach.
Exposure of 0.1 to 1 μmol/mol produces headaches, burning eyes and
irritation to the respiratory passages. Even low concentrations of ozone
in air are very destructive to organic materials such as latex,
plastics and animal lung tissue.
Seaside air was once considered to be healthy because of its "ozone" content.
It is now recognised that the smell in reality is released from bacteria
following the breakdown of species from rotting seaweed! (dimethyl sulfoxide, DMS).
Birds appear to be attracted to the smell since for them it indicates a
plankton bloom, and therefore the presence of fish feeding on the marine plants.
Ozone may be formed from O2 by electrical discharges and by
action of high energy electromagnetic radiation. Unsuppressed arcing
breaks down the chemical bonds of the atmospheric oxygen surrounding
the contacts [O2 → 2O]. Free radicals of oxygen in and
around the arc recombine to create ozone [O3]. Certain electrical
equipment generate significant levels of ozone. This is especially true of
devices using high voltages, such as ionic air purifiers, laser printers,
photocopiers, tasers and arc welders. Electric motors using brushes can
generate ozone from repeated sparking inside the unit. Large motors that
use brushes, such as those used by elevators or hydraulic pumps, will
generate more ozone than smaller motors. Ozone is similarly formed in the
Catatumbo lightning storms phenomenon on the Catatumbo River in Venezuela,
which helps to replenish ozone in the upper troposphere. It is the world's
largest single natural generator of ozone. The lightning originates from
a mass of storm clouds at a height of more than 5 km,
and occurs during 140 to 160 nights a year, 10 hours per day and up to 280
times per hour.
Ozone-Oxygen cycle
Ninety percent of the ozone in the atmosphere sits in the stratosphere,
the layer of atmosphere between about 10 and 50 kilometers altitude.
The ozone layer is mainly found in the lower portion of the stratosphere,
from approximately 20 to 30 kilometres (12 to 19 mi) above Earth, though the
thickness varies seasonally and geographically. Here the concentration of
O3 varies from two to eight ppm which is much larger than the
average ozone concentration in the Earth's atmosphere which is only about
0.3 parts per million.
The natural level of ozone in the stratosphere can be considered to be a
result of a balance between sunlight that creates ozone and chemical
reactions that destroy it.
Creation: photolysis of an oxygen molecule by high
energy UV light splits it into two oxygen atoms. The wavelength in the UV
needed to achieve this can be estimated from the O=O Bond Energy of
498 kJmol-1.
By dividing this by 6.022 * 1023 bonds/mol the
Energy per bond is found to be 8.27 * 10-19 J/bond.
The wavelength required for photolysis can be evaluated from E= hc / λ
λ= hc /E = (6.626 * 10-34 Js x 3 * 108 m/s) / ( 8.27 * 10-19 J)
so λ= 240 nm, i.e. visible light cannot break the O=O bond, but UV-C
light with energy > 240 nm can break the O=O bond.
{UV ranges are: UV-A (400-315 nm), UV-B (315-280 nm), and UV-C (280-200 nm).}
O2 + hν → 2O
Each oxygen atom then rapidly combines with an oxygen molecule to form an
ozone molecule:
O + O2 → O3
The ozone-oxygen cycle: the ozone molecules formed by the
reaction above absorb radiation again having an appropriate wavelength in the UV.
The triatomic ozone molecule becomes diatomic molecular oxygen plus a free
oxygen atom:
O3 + hν (240-310 nm) → O2 + O
The atomic oxygen produced quickly reacts with another oxygen molecule to reform ozone:
O + O2 → O3 + K.E.
where "K.E." denotes the excess energy of the reaction which is manifested
as extra kinetic energy. These two reactions form the ozone-oxygen cycle,
in which the chemical energy released when O and O2 combine is converted
into kinetic energy of molecular motion. The overall effect is to convert
penetrating UV-B light into heat, without any net loss of ozone. This cycle
keeps the ozone layer in a stable balance while absorbing 97-99% of the
Sun's medium-frequency ultraviolet light (from about 200 nm to 315 nm
wavelength) and preventing it from reaching the Earth's surface,
to the benefit of both plants and animals.
This reaction is one of two major sources of heat in the stratosphere (the other
being the kinetic energy released when O2 is photolyzed into
O atoms).
Removal: reaction of an oxygen atom with an ozone molecule
leads to production of two oxygen molecules:
O3 + O. → 2 O2
and if two oxygen atoms meet, they can react to form one oxygen molecule:
2 O. → O2
The overall amount of ozone in the stratosphere is determined by a balance
between production by solar radiation and removal. The removal rate is slow,
since the concentration of O atoms is very low. Certain free radicals, the
most important being hydroxyl (OH), nitric oxide (NO) and atoms of
chlorine (Cl) and bromine (Br), catalyze the recombination reaction,
leading to an ozone layer that is thinner than it would be if the
catalysts were not present.
Most of the OH and NO are naturally present in the stratosphere, but
human activity, especially emissions of chlorofluorocarbons (CFCs) and
halons, has greatly increased the Cl and Br concentrations, leading to
ozone depletion. Each Cl or Br atom can catalyze tens of thousands of
decomposition reactions before it is removed from the stratosphere.
Follow the
Ozone Hole Watch at NASA and see the
ozone depletion wikipedia page.
Ozone depletion
Ozone depletion describes two distinct but related phenomena observed
since the late 1970s: a steady decline of about 4% per decade in the total
volume of ozone in the ozone layer, and a much larger springtime decrease
in stratospheric ozone over Earth's polar regions. Note that the Antarctic
would be in darkness (no sunshine) for the winter months, sunshine returning in
springtime (September). The latter phenomenon is referred to as the ozone hole.
The most important process in depletion is catalytic destruction of ozone by
atomic halogens. The main source of these halogen atoms in the stratosphere
is photodissociation of man-made halocarbon refrigerants, solvents,
propellants, and foam-blowing agents (CFCs, HCFCs, freons, halons),
substances that are referred to as ozone-depleting substances (ODS).
In the simplest example of such a cycle, a chlorine atom reacts with an
ozone molecule, taking an oxygen atom with it (forming ClO) and leaving a
normal oxygen molecule. The chlorine monoxide (ClO) produced can react with
a second molecule of ozone to yield another chlorine atom and two
molecules of oxygen. These gas-phase reactions can be written such that
in the first step a chlorine atom changes an ozone molecule to ordinary oxygen:
Cl. + O3 → ClO + O2
The ClO generated can then destroy a second ozone molecule and
recreate the original chlorine atom, which can repeat the first reaction
and continue a cycle to destroy ozone.
ClO + O3 → Cl. + 2 O2
In theory, a single chlorine atom could keep on destroying ozone
(acting as a catalyst) for up to two years (the time scale for transport
back down to the troposphere) were it not for reactions that remove Cl by
forming reservoir species such as hydrogen chloride (HCl).
In practise, the average chlorine atom reacts with 100,000 ozone
molecules before it is removed from the catalytic cycle.
On a per atom basis, bromine is even more efficient than chlorine at
destroying ozone, but at present there is much less bromine in the atmosphere.
Both chlorine and bromine significantly contribute to the overall depletion
of ozone.
The Montreal Protocol on Substances that Deplete the Ozone Layer
(a protocol to the Vienna Convention for the Protection of the Ozone Layer)
is an international treaty designed to protect the ozone layer by phasing
out the production of numerous substances that are responsible for ozone
depletion. It was agreed on in 1987, and entered into force on January 1, 1989,
with numerous revisions since then.
The bans on the production of CFCs, halons, and other ozone-depleting
chemicals such as carbon tetrachloride and trichloroethane have led to the
expectation of a recovery of the ozone layer to 1980 values somewhere between
2050 and 2070. This was the estimate given in a summary document of the
Scientific Assessment of the Ozone Depletion 2014 published by the UN Environment
Programme (UNEP) and the UN World Meterological Organization (WMO).
Among the key findings of the report were that the authors noted that what
happens after 2050 will then largely depend on concentrations of CO2,
methane and nitrous oxide - the three main long-lived greenhouse gases
in the atmosphere.
Ozone is much less stable than the diatomic allotrope O2,
breaking down in the lower atmosphere to normal dioxygen. The O - O distances
in O3 are 127.2 pm and the O - O - O angle is 116.78°. The
bonding can be expressed as a resonance hybrid with a single bond on one side
and double bond on the other producing an overall average bond order of 1.5
for each side.
Ozone is a powerful oxidant (far more so than dioxygen) and has many
industrial and consumer applications related to oxidation. This same high
oxidizing potential, however, causes ozone to damage mucous and respiratory
tissues in animals, and also tissues in plants, above concentrations of
about 100 ppb. This makes ozone a potent respiratory hazard and pollutant
near ground level.
Ozone oxidizes nitric oxide to nitrogen dioxide:
NO + O3 → NO2 + O2
This reaction is accompanied by chemiluminescence. The NO2 can be further
oxidized:
NO2 + O3 → NO3 + O2
The NO3 formed can react with NO2 to form N2O5.
Ozone does not react with ammonium salts, but it oxidizes
ammonia to ammonium nitrate:
2 NH3 + 4 O3 → NH4NO3 + 4 O2 + H2O
Ozone reacts with carbon to form carbon dioxide, even at room temperature:
C + 2 O3 → CO2 + 2 O2
On increasing the pressure of dioxygen at room temperature, the "solid
oxygen β-phase" undergoes phase transitions to the δ-phase at
9 GPa and the ε-phase at 10 GPa. Its volume decreases significantly,
and it changes colour from blue to deep red. The structure of this red
ε-phase, determined in 2006, is based on an O8 cluster
(see structure above). It was confirmed that this structure is formed up to 96 GPa.
The box-like cluster is an unusual conformation first recognised for oxygen,
and as yet has not been experimentally or theoretically reported for any
other diatomic molecule.
Liquid oxygen is already used as an oxidant in rockets, and it has been
speculated that red oxygen could make an even better oxidant, because of
its higher energy density.
Hydrogen peroxide (H2O2) is the simplest peroxide
(a compound with an oxygen-oxygen single bond) and in its pure form is
a colourless liquid that is slightly more viscous than water. It is a
strong oxidizer and is used as a bleaching agent and disinfectant.
For safety reasons it is normally used as an aqueous solution, also colourless.
For consumers, it is usually available from pharmacies at 3 and 6 wt%
concentrations. The concentrations are sometimes described in terms of the
volume of oxygen gas generated; one milliliter of a 20-volume solution
generates twenty milliliters of oxygen gas when completely decomposed.
For laboratory use, 100 volume, 30 wt% solutions are the most common.
Concentrated H2O2, or 'high-test peroxide' is a
reactive oxygen species and has been used as a propellant in rocketry.
Diluted H2O2 (between 1.9% and 12%) mixed with
ammonium hydroxide has been used to bleach human hair. The chemical's
bleaching property lends its name to the phrase "Hollywood peroxide blonde".
Hydrogen peroxide can be used for tooth whitening and when mixed with baking
soda and salt forms a recipe for home-made toothpaste.
Discovery
Hydrogen peroxide was first described in 1818 by
Louis Jacques Thénard, who produced it by treating barium peroxide
with nitric acid. An improved version of this process used hydrochloric acid,
followed by addition of sulfuric acid to precipitate the barium sulfate
byproduct. Thénard's process was used from the end of the 19th century
until the middle of the 20th century.
Pure hydrogen peroxide was long believed to be unstable as early attempts
to separate it from the water, which is present during synthesis, all failed.
This instability was due to traces of impurities (transition metals salts)
which catalyze the decomposition of the hydrogen peroxide. Pure hydrogen
peroxide was first obtained in 1894 - almost 80 years after its discovery
- by Richard Wolffenstein, who produced it via vacuum distillation.
Determination of the molecular structure of hydrogen peroxide proved to
be very difficult. In 1892 the Italian physical chemist Giacomo Carrara
(1864-1925) determined its molecular weight by freezing point depression,
which confirmed that its molecular formula was H2O2.
At least half a dozen hypothetical molecular structures seemed to be
consistent with the available evidence. In 1934, the English mathematical
physicist William Penney and the Scottish physicist Gordon Sutherland
proposed a molecular structure for hydrogen peroxide which was very similar
to the presently accepted one and which subsequent evidence cumulatively
proved to be correct.
The structure of hydrogen peroxide
H2O2 - gas phase
(O-O 147.4 pm)
|
H2O2 - solid phase
(O-O 145.8 pm)
|
Although the O-O bond is a single bond, the molecule has a relatively high
barrier to rotation of 29.45 kJmol-1 (2460 cm-1);
for comparison, the rotational barrier for ethane is just 12.5 kJmol-1.
The increased barrier is thought to be due to the repulsion between the
lone pairs of the adjacent oxygen atoms.
The molecular structures of gaseous and crystalline H2O2
are significantly different. This difference is attributed to the effects of
hydrogen bonding, which is absent in the gaseous state.
The anthraquinone process is a process for the production of hydrogen
peroxide, which was developed by BASF. The industrial production of
hydrogen peroxide is based on the reduction of oxygen with hydrogen,
by the direct synthesis from the elements. Instead of hydrogen itself,
however, a 2-alkyl-anthrahydroquinone (generated from the corresponding
2-alkyl-anthraquinone by catalytic hydrogenation with palladium) is used.
Oxygen and the organic phase react to give hydrogen peroxide and the
anthraquinone can be recycled.
The hydrogen peroxide is then extracted with water in a second step
and separated by fractional distillation. The anthraquinone
thus acts as a catalyst with the overall reaction given by the equation:
H2 + O2 → H2O2
If ozone is used instead of oxygen,
dihydrogen trioxide, H2O3 can be produced by this method.
Decomposition
Hydrogen peroxide is thermodynamically unstable and decomposes to form
water and oxygen with a ΔH⦵ of -98.2 kJmol-1
and a ΔS⦵ of 70.5 Jmol-1K-1.
2 H2O2 → 2 H2O + O2
The rate of decomposition increases with rising temperature, concentration
and pH, with cool, dilute, acidic solutions showing the best stability.
Decomposition is catalysed by various compounds, including most transition
metals and their compounds (e.g. manganese dioxide, silver, and platinum).
Certain metal ions, such as Fe2+ or Ti3+, can cause
the decomposition to take a different path, with free radicals such as
(HO.) and (HOO.) being formed.
The decomposition of hydrogen peroxide liberates oxygen and heat; this
can be dangerous as spilling high concentrations of hydrogen peroxide on
an inflammable substance can cause an immediate fire.
Metal oxides, peroxides and superoxides
When the group 1 metals are heated in an excess of air or in O2,
the principal products obtained depend on the metal:
lithium oxide, Li2O, sodium peroxide, Na2O2,
and the superoxides KO2, RbO2 and CsO2.
4Li + O2 → 2Li2O oxide formation
2Na + O2 → Na2O2 peroxide formation
K + O2 → KO2 superoxide formation
The superoxides and peroxides contain the paramagnetic [O2]-
(μ ~ 1.73 BM) and diamagnetic [O2]2- ions
respectively. See the MO diagram for O2 above for comparison.
All of the monoxides are known, M2O, and the heavier peroxides
and superoxides will decompose to form the oxides. They are all ionic and
strong bases with the basicity increasing down the group.
O2- + H2O(aq) → 2 OH- (aq)
Sodium peroxoborate
is a solid peroxygen compound with
exceptional storage stability and no shock sensitivity. It is cheap and
readily available, being produced mainly as a solid ingredient of domestic
washing formulations, in which it acts as sources of H2O2
in solution for stain bleaching. World annual production in 1995 was
approximately 750,000 tonnes, and its use in washing powders dates back to 1907
with Henkel's original "Persil" product in Germany.
Sodium peroxoborate has the empirical formula "NaBO3.xH2O".
Two forms that are commercially available correspond stoichiometrically
to x = 1 or 4, and are known as the "monohydrate" and "tetrahydrate',
respectively. Structurally, however, sodium peroxoborate was shown in
1961 to be the disodium salt of a 1,4.-diboratetroxane dianion.
Hence, the "monohydrate" really corresponds to the anhydrous salt, and
the "tetrahydrate" to a hexahydrated form of it. The monohydrate form
dissolves better than the tetrahydrate and has higher heat stability;
it is prepared by heating the tetrahydrate. The compound exists as a dimer
as shown below.
The reagent offers low toxicity and a long shelf life. Sodium peroxoborate
is a useful reagent in
synthetic chemistry as a substitute for the unstable,
highly concentrated hydrogen peroxide solutions that can pose a significant
explosion hazard and are not commercially available.
Persil and the structure of Na
2B
2(O
2)
2(OH)
4.6H
2O
Return to the
course outline
or move on to Lecture 5: Structure of the elements
(Groups 1 and 2 metals,
Boron, Carbon
and Phosphorus, Sulfur).
References
Much of the information in these course notes has been sourced from Wikipedia under
the Creative Commons License.
'Inorganic Chemistry' - C. Housecroft and A.G. Sharpe, Prentice Hall, 4th Ed.,
2012, ISBN13: 978-0273742753, pps 24-27, 43-50, 172-176, 552-558, 299-301, 207-212
'Basic Inorganic Chemistry' - F.A. Cotton, G. Wilkinson and P.L.
Gaus, John Wiley and Sons, Inc. 3rd Ed., 1994.
'Introduction to Modern Inorganic Chemistry' - K.M. Mackay, R.A.
Mackay and W. Henderson, International Textbook Company, 5th Ed., 1996.
This work is licensed under a Creative Commons
Attribution-ShareAlike 3.0 Unported License.
Return to Chemistry,
UWI-Mona, Home Page
Created and maintained by Prof. Robert J.
Lancashire,
The Department of Chemistry, University of the West Indies,
Mona Campus, Kingston 7, Jamaica
Created November 2014. Links checked and/or last
modified 17th February 2015.
URL http://wwwchem.uwimona.edu.jm/courses/CHEM1902/IC10K_MG_oxygen.html