Dioxygen uptake by Co(salen)
Naturally occuring dioxygen carriers and storage proteins contain
a transition metal ion to which dioxygen can reversibly bind, eg.
Iron (myoglobin,Mb, haemoglobin, Hb) or Copper (haemocyanin). In
this experiment a simple cobalt complex will be prepared which
also reversibly binds dioxygen. Many complexes of this type have
been used as "models" to aid in the understanding of how the
proteins function.
When Co(salen) was first prepared in 1933, it was observed that
the red-brown crystals darkened on exposure to air. However, it
was not until five years later that it was established that the
colour change was due to reversible uptake of dioxygen. H2salen
(I) is a Schiff-base ligand formed by the condensation of two
molecules of salicylaldehyde with 1,2-diaminoethane
(ethylenediamine).
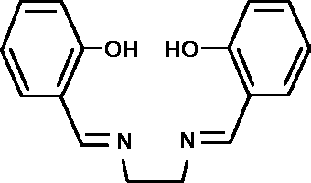
(II)
In 1944, it was found that different crystalline forms existed
depending on the solvent used in the preparation or for
recystallisation and that these had varying capacity for
oxygenation in the solid state. This variation in oxygenation has
been related to the presence of voids in the crystal lattice,
sufficient to allow the passage of molecular oxygen. This
suggestion is supported by the X-ray crystal structure
determination of the so-called "inactive" form which shows that
the structure consists of di meric units [Cosalen]2, (II).
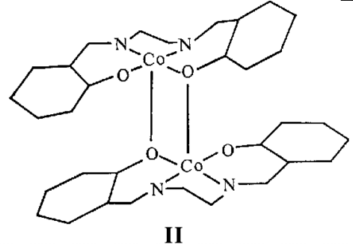
(II)
The active forms of Cosalen are presumed to contain dimeric
units with open lattices packing relative to the inactive form.
One form (III) has one Co atom directly above the other.
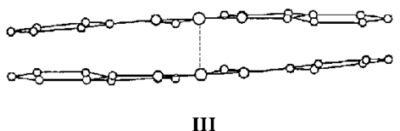
(III)
The importance of solid-state packing effects in determining
oxygenation ability is further indicated by kinetic studies of
dioxygen uptake at the crystal surfaces of the different forms of
Co(salen). Measurements involving different temperatures and
pressure conditions have shown that, following an induction
period and after attainment of equilibrium, the kinetics of
dioxygen absorption at the surface were no longer important and
the uptake was controlled only by the rate of diffusion of
dioxygen into the crystal.
In solution, it has been found that, depending on the solvent, in
the absence of dioxygen, the cobalt(II) may be four, five or six
coordinate. For example, in a strongly coordinating solvent such
as pyridine, both [Co(salen).pyr] and [Co(salen).2pyr] exist,
whilst in chloroform, the major species appears to be Co(salen).
Irrespective of the solvent, the rate of dioxygen uptake appears
to be similar, however the product obtained may be a 1:1 (IV) or
a 2:1 (V)(oxygen bridged) complex.
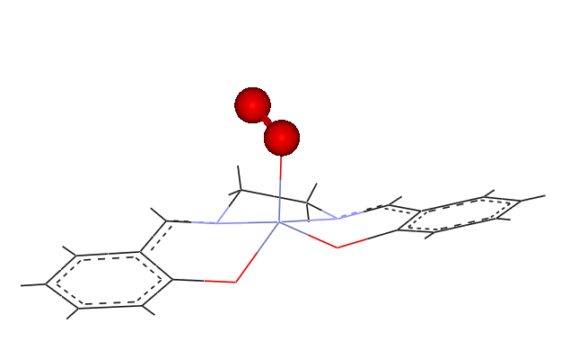
(IV)
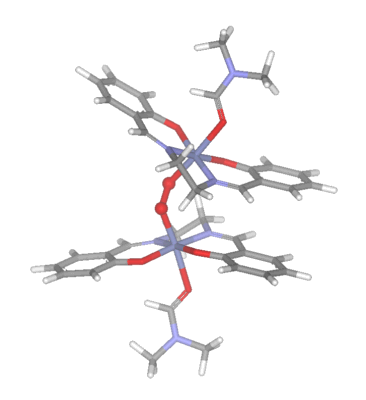
(V)
In this experiment, the inactive form of Co(salen) is
prepared. The uptake of dioxygen is then investigated for the
complex in DMSO solution to establish whether a 1:1 or a 2:1
complex is formed under these conditions.
Preparations.
H2salen
To a solution of salicylaldehyde (2.1 cm3) in 25 cm3 boiling
ethanol is added 1,2-diaminoethane (0.7 cm3). The reaction
mixture is thoroughly stirred for 3-4 minutes and the solution
then left to cool in an ice-bath. The bright yellow flaky
crystals are filtered under suction and washed with a small
volume of ice-cold ethanol, then air-dried. The yield and the
melting-point should be recorded.
Cosalen
This preparation is sensitive to air, so should either be
performed whilst flushing a stream of nitrogen gas through the
flask or under vacuum. The arrangement for working under reduced
pressure is given below.
Dissolve H2salen (1.6 g) in 60 cm3 of ethanol at
60-70°ree;C in a
side-arm flask fitted with a clamp. The side-arm is connected to
an aspirator. Upon dissolution of the ligand, quickly add
Cobalt(II) acetate tetrahydrate (1.25 g) in 7 cm3 of
ethanol:water mixture, while swirling the flask. Immediately
stopper the flask and evacuate through the side-arm for a short
while. NOTE: The flask MUST be securely clamped since the ethanol
mixture has a tendency to bump vigorously. Be careful not to draw
off the bulk of the ethanol and clamp the side-arm once reduced
pressure has been established. Continue heating with periodic
swirling for 1-2 hours.
During this period, the initially formed brown "active" complex
slowly changes to the brick-red "inactive" complex. Once this has
occurred, cool the solution to room temperature, collect the
crystals on a sintered glass filter funnel (in air) and wash
three times with 7 cm3 of ice-cold ethanol. Dry in a dessicator,
then record the yield.
An IR spectrum from a typical
student preparation is available.
Dioxygen Absorption by Co(salen) in DMSO
Procedure
Accurately weigh out a sample of Co(salen) which has been finely
ground (between 0.05 and 0.1 g) and place it in a side-arm test
tube. Transfer DMSO (approximately 5 cm3) to a small beaker and
bubble oxygen through it for a few seconds. (CAUTION: although
DMSO is not itself poisonous, it is readily absorbed by the skin
and can easily carry other compounds through the skin with it).
Now transfer this DMSO to a small test tube that can fit inside
the side-arm test tube and lower the tube carefully inside
without spillage.
Connect up the apparatus as shown in the diagram, so that the
movable arm reservoir can be adjusted to bring the water level of
the graduated tube near the bottom.
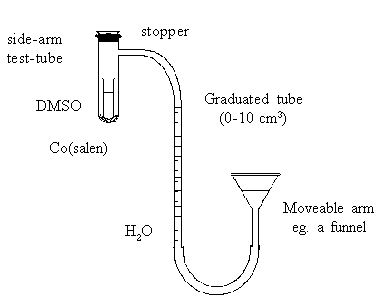
Flush the side-arm tube with a gentle stream of oxygen. Insert
a tightly fitting rubber stopper in the mouth of the tube. Adjust
the movable arm to make the water levels equal in both sides (ie
ensuring that the pressure within the apparatus is atmospheric).
Record the water level in the graduated tube.
Finally, carefully invert the side-arm tube (holding near the
stopper to minimise heating by the hand) and record the time. The
DMSO should be allowed to dissolve the Co(salen) but not be
spilled into the side-arm. As oxygen is absorbed, the water level
in the graduated tube begins to rise. Note the changes occurring
in the tube. Continue shaking until no further change in water
level occurs (taking a reading every two minutes for 20 minutes
is usually sufficient). Adjust the moveable arm before each
reading so that the water levels in the tubes are again equal.
Draw a graph of volume changes versus time and extrapolate to
estimate the overall total oxygen uptake. From this volume change
at room temperature and atmospheric pressure, the number of mole
of dioxygen absorbed per mole of Co(salen) can be calculated.
Electron Paramagnetic Resonance
Suppose that an electronic spin system of S=0.5 is brought under
the influence of a static magnetic field H. The energy state of
the system would split into two, Ms=+0.5 and Ms=-0.5, owing to
the interaction of the spin system with the magnetic field. The
energy gap between the two states is ΔE = 2βH,
where β is in Bohr Magnetons, the unit of electron spin moment.
The majority of the
spins of the system are in the lower energy state. When an
electromagnetic wave of frequency v is applied to this system and
a condition ΔE=hν is satisfied, an excitation from the lower to
the upper level occurs. As a result, a fraction of the energy of
the electromagnetic wave will be absorbed by this system. For a
free unpaired spin then,
ΔH= hv=2βH
Usually a microwave of 3.2 cm wavelength (called the X-band) is
used for EPR. Then v ~ 9200 MHz. By putting explicit values for Β
and h, we find that H =3200 gauss. The resonance condition is
more generally expressed using the g value ie,
ΔH= hv=gβH
where g is called the spectroscopic splitting factor and is equal
to 2.00229 for a free radical. Values of g for a formal orbitally
singlet ground state can be expressed by;
g=2(1-nλ/Δ)
where λ is the effective spin-orbit coupling constant, Δ is the
energy gap between the ground level and the excited level in
question, and n is a constant depending on the two levels
concerned. Thus, the g value may afford information about the
effective value of λ and the energy gap Δ. The former has been
interpreted to give a measure of covalency of the bond between
the central metal ion and the ligands.
The g value can vary from one direction to another ie, if x-, y-
and z-axis are defined in a complex, gx, gy and gz may not be the
same. In a strictly octahedral complex they should be equal
(isotropic), but if the symmetry is lowered to tetragonal then
gx=gy =gz. For less than axial symmetry then gx=gy=gz. This means
that the fundamental symmetry of the ligand field may be deduced
from the shape of the EPR spectrum.
Co(II) has a d7 configuration and for low spin complexes S=0.5. l
is negative and generally gx>gy>gz. To complicate the
appearance, the hyperfine interaction with the nucleus of the Co
atom, which has I=7/2, splits each band into eight peaks (2I+1).
In most cases, Ax and Ay are small and the hyperfine structures
on gx and gy are not well defined, while the hyperfine on gz
gives well separated peaks.
A rough estimate of g and A can be obtained from the spectrum,
but for precise values computer simulations need to be performed.
The curves shown below are from computer simulation exercises and
also display "stick spectra" indicating the positions for the
measurement of the A values.
1) Co(salen) in pyridine
Note that the best resolved peaks show superhyperfine
splitting due to the presence of a N from one coordinated
pyridine group (IN=1). The g values obtained were 2.464, 2.244
and 2.024.
2) A simulation of the dioxygen adduct of a Co(II) substituted
Salen complex.
Note the expanded scale. The g values obtained here were
1.994, 2.010 and 2.081.
Questions
1. Sketch the spectra and carefully label the positions of the g
and A values. Calculate the A values from the separations of the
stick spectra.
2. The relative sizes of the A values has been used to predict
the occupancy of the unpaired electron on the Cobalt in the
dioxygen complex. Explain
3. Similar EPR spectra have been obtained from cobalglobin
(obtained by replacing Fe by Co in Haemoglobin). On this basis,
it has been suggested that the Cobalt model compounds are
electronically good models for Hb. What other factors need to be
considered before these complexes can be considered good models
for all aspects of Hb.
References
General
F.A. Cotton and G. Wilkinson, "Advanced Inorganic Chemistry",
Fifth Edition,Wiley-Interscience, New York, 1988, pages 735-738.
T Appleton, J. Chem. Educ., 54, 1977, 443.
X-Ray Structures
N.G. Vannerburg, Acta Cryst., 18, 1965, 449.
S. Bruckner, M. Calligaris, G. Nardin and L. Randaccio, Acta
Cryst., B2, 1969, 1971.
R. Delasi, S.L.Holt and B. Post, Inorg. Chem., 10, 1971, 1498.
W.P. Schaefer and R. E. March, Acta Cryst., B25, 1969, 1675.
M. Calligaris, G. Nardin, L. Randaccio and A. Ripamonti, J. Chem, Soc., A
(1970), 1069.
Electron Paramagnetic Resonance
T.D. Smith and J.R. Pilbrow, Coord. Chem. Rev., 39, 1981, 295.
R.J. Lancashire, T.D. Smith and J.R. Pilbrow, J. Chem Soc. Dalton
Trans., 1979, 66.
Return to Chemistry, UWI-Mona,
Home Page
Copyright © 2005 by Robert John
Lancashire, all rights reserved.
Created and maintained by Prof. Robert J.
Lancashire
The Department of Chemistry, University of the West Indies,
Mona Campus, Kingston 7, Jamaica.
Created November 1996. Links checked and/or last
modified 8th November 2005.
URL
http://wwwchem.uwimona.edu.jm/lab_manuals/c31lex2.html